The Neutrino Hunter
In hot pursuit of the elusive neutrino, physicist Janet Conrad ’85 seeks clues to some of science’s deepest mysteries.
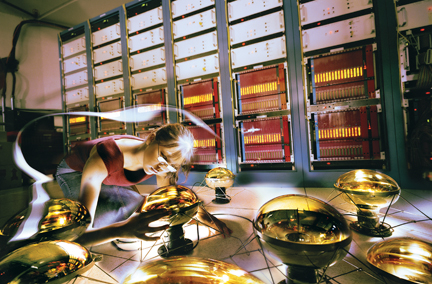
Jasmine Ma, a graduate student working on the MiniBooNE experiment, inspects photo tubes that detect light from neutrino interactions.
If particles had personalities, what a family they would be! Quarks are so cliquish that they travel only in groups of three and are never seen alone. Flashy photons, all style and no substance, travel everywhere at the speed of light. Businesslike electrons bind atoms to one another and power all our electronic gadgets.
One kind of particle wanders through the middle of the hubbub yet somehow remains detached from it all. These particles are everywhere, and yet they are nearly undetectable. They cannot be contained or steered—and they pass through walls as if they weren’t there. They’re called neutrinos, and they may be the most important particle in the universe. Every time a photon of light is created, a neutrino is created as well. It’s safe to say that if the universe had no neutrinos, we wouldn’t be here either. And yet they are the least understood subatomic particle around.
“That’s the reason I got interested in neutrinos,” says Janet Conrad ’85, a professor of physics at Massachusetts Institute of Technology. Not only are they next to impossible to catch, but they also have a chameleon-like ability to change identities—an ability that physicists were not even aware of until about 10 years ago. Conrad has recently discovered evidence for the fourth and most exotic of the neutrino’s multiple personalities, the “sterile neutrino.” If it is real, it could be a clue to some of the deepest mysteries in physics, such as the nature of dark matter and whether extra dimensions exist.
WONDER AND JOY
Of course, particles don’t really have personalities. But people do. And Conrad is almost the opposite of the neutrino, the “little neutral one.” She is full of enthusiasm and loves to tell the public about her work. In her last few years at Columbia University, where she was on the faculty for 13 years before moving to MIT in 2008, she regularly taught the proverbial “physics for poets” course and drew upwards of 100 students to her classes.
“Physicists have made physics seem so esoteric, because it makes them look smart,” Conrad says. “The irony of my work is that you can learn everything you need to understand neutrino oscillations by the end of your sophomore year at Swarthmore.”
Appropriately, Conrad’s office looks more like a toy shop than an academic office. In various corners you can find prisms, Slinkies, water rockets, tuning forks, and a set of mirrors that create the image of a shell floating in the air above her desk. She has a collection of hand-blown Crookes radiometers—a little lightbulb-shaped device with a vane inside that spins when light strikes it. “When I walk in the office and turn on the light, they immediately start turning,” she says. “It’s amusingly welcoming.”
The toys are there partly for fun and partly because they demonstrate physical principles—the same basic principles about wave motion and conservation of energy that underlie her seemingly more esoteric research on neutrinos. “To me, physics is about really wonderful and joyful things, like toys. And very accessible things like blue skies and waves,” she says. “That’s why I find it so sad when people say my field is inaccessible. It really isn’t at all.”
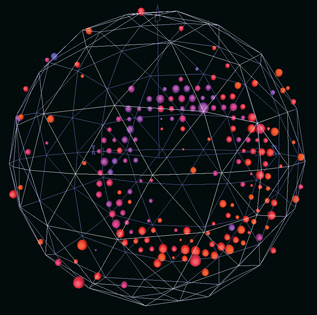
A ring of light, registered by some of the more than 1,000 detectors in the experiment, indicates the collision of a muon neutrino with an atomic nuclei.
IN SEARCH OF THE WILD NEUTRINO
To detect a neutrino, you need two things: a prolific neutrino source and a detector. Sources are easy to come by. Any nuclear reactor will do, because neutrinos are emitted in the process of radioactive decay. A particle accelerator will also work, or even the sun—the great neutrino factory in the sky. The hard part to arrange is the detector.
Because they are so small and have no electric charge, neutrinos almost never interact with matter. But if you direct a few billion neutrinos at a sufficiently massive target, a few of them will happen to hit a proton head-on and release a flash of light. So the typical neutrino detector is an enormous tank of liquid, surrounded by light detectors. They are usually placed underground, so that the earth’s crust can filter out other particles, such as cosmic rays, that might be mistaken for neutrinos. The first successful neutrino detector, in 1956, was a 200-liter tank of mineral water that caught a whopping three neutrinos per hour. The largest neutrino detector, in Japan, contains 50,000 tons of water. At the Department of Energy’s Fermi National Laboratory in Battavia, Ill., Conrad’s experiment MiniBooNE—short for Booster Neutrino Experiment—uses a comparatively modest 800 tons (or 30 tank-loads) of mineral oil.
Nowadays, physicists don’t just want to detect neutrinos—they want to understand their amazing mutability. Neutrinos come in at least three “flavors,” called electron, muon, and tau neutrinos. But these flavors do not stay fixed. An experiment in 2001 at the Sudbury Neutrino Observatory (SNO), located deep in a Canadian nickel mine, measured neutrinos coming all the way from the Sun. It found only one third as many electron neutrinos as expected. There were two possibilities: either the Sun was not making as many neutrinos as we thought—or the other two thirds of the neutrinos were there, but they had changed into muon or tau neutrinos (which the SNO was not equipped to detect). It was as if you went out to the store and bought a box of chocolate ice cream, and by the time you got home it had turned into Neapolitan.
This might seem like a crazy idea, but theorists knew already that it was a possibility, provided that neutrinos had mass. The reason, Conrad says, is a well-known phenomenon called wave interference. You can see wave interference any time you look at a prism or an iridescent material, such as an oil slick. White light consists of waves of many colors or frequencies, which usually move at the same velocity. But a prism causes different colors of light to travel at different rates. The waves are no longer synchronized, and they “interfere” with each other, so that from one direction the refracted light looks red, while from another it looks blue or green.
The changing flavors of the neutrino are exactly like the changing colors of light. In theory, each neutrino is actually a trio of matter waves, which travel at different rates because they have different masses. Like the light reflected off an oil slick that looks alternately red, green, and blue, the neutrino will “look” in some places like an electron neutrino, in other places like a muon neutrino, and so on. To return to the ice cream metaphor, the chocolate ice cream doesn’t just change to Neapolitan. It changes to vanilla, and strawberry, and every possible combination (including Neapolitan), many times every second. The flavor you happen to measure with your detector depends on when you catch it.
This “neutrino oscillation” was a surprising discovery, but only because physicists had never before found any evidence that neutrinos have mass. The SNO experiment could be explained with only a slight modification to existing theory (making the neutrino’s mass nonzero); it did not require a complete rethinking of neutrino physics.
MiniBooNE complicated this picture. It was intended to test an anomalous result that had been found at the Los Alamos National Laboratory by Conrad’s collaborator, Bill Lewis, which seemed to show that the trio must actually be a quartet. The fourth member (the “sterile neutrino”) would have to be the most introverted neutrino yet. It would be an excellent candidate for what cosmologists call “dark matter,” a mysterious form of matter that seems to make up about a quarter of our universe, but which we know about only through its gravitational effects.
The results from MiniBooNE did not confirm the Los Alamos experiment—but they did detect an excess of low-energy neutrinos (369 instead of the expected 273 over a six-year period). The excess may have to do with sterile neutrinos or some other physics beyond the “Standard Model.” Or it could be an experimental artifact. A stray photon can sometimes be mistaken for the flash of light produced by a neutrino. “One of them is a little fuzzier than the other, but in the end fuzz is fuzz—it’s hard to tell the difference,” Conrad says.
One of Conrad’s former students, Bonnie Fleming of Yale University, is currently developing a new experiment, called MicroBooNE, which should resolve the ambiguity. For the first time it would detect actual neutrino tracks, rather than single flashes of light. It will also have pinpoint accuracy compared to MiniBooNE. To tell the difference between a fuzzy flash and a sharp flash, it helps to have a sharp pair of glasses.
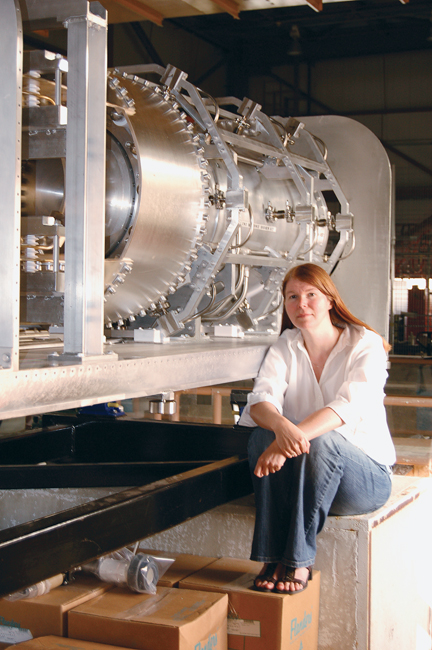
Although fewer than 20 percent of U.S. physics doctorates go to women, Janet Conrad's research teams have been half men and half women.
MENTOR AND ROLE MODEL
Conrad was upset when the then-president of Harvard University, Lawrence Summers, suggested in 2005 that the low proportion of women in some sciences might have something to do with their innate abilities (read sidebar). In fact, a look at Conrad’s research groups shows that neutrinos don’t care about gender. At MiniBooNE, about a third of the experimenters are women.
It’s easy to see why students of any gender would gravitate toward Conrad. “She’s a really dedicated adviser,” Fleming says. “She teaches you how to be a good scientist.” According to Boris Kayser, a neutrino physicist at Fermilab, “Apart from [teaching] techniques and skills, she looks after the careers of her protégés and teaches them what they need to do to succeed.”
In part, she leads by example. It is very unusual for a junior professor, as Conrad was in 2001 when MiniBooNE got started, to organize a major experiment at a national lab. Fleming got to see firsthand how it is done, from hiring people to managing them and finding the right roles for them. Now Fleming is taking on the same role for MicroBooNE. “It’s really exciting to go from a mentoring relationship with Bonnie to working with her as a colleague,” says Conrad.
Conrad believes that women in physics do undergo more scrutiny than men. “Being a woman in physics is like being an expatriate,” she says. She spent a year in England after graduating from Swarthmore, and she says that she was “held responsible for everything America did, such as Reagan bombing Libya.” But, she adds, “An expatriate chooses to be an expatriate for a reason: They love the opportunities. I love being in physics, and the opportunities it gives me.”
Dana Mackenzie ’79 always wanted to be a writer when he was a child. But he loved mathematics, too, so he took a 20-year detour: majoring in math at Swarthmore, getting a Ph.D. at Princeton, and teaching at Duke University and Kenyon College. Since 1997, he has been a freelance writer, specializing in math and science. His first book, The Big Splat, or How Our Moon Came to Be, was published in 2003.